Akinsemolu A.A.
Adeyemi Federal University of Education, Ondo State, Nigeria
Corresponding Author Email: akinsemoluaa@aceondo.edu.ng
Abstract
Green microbial technology offers a transformative, sustainable approach to addressing the escalating challenge of environmental pollution. This chapter examines how the natural metabolic capacities of microorganisms can be harnessed for bioremediation, transforming hazardous contaminants such as petroleum hydrocarbons, heavy metals, and pesticides into less harmful compounds. Traditional degradation processes, both aerobic and anaerobic, are enhanced by modern advances in genetic engineering and synthetic biology. Customised microbial strains and engineered consortia have demonstrated improved pollutant breakdown and increased resilience under variable environmental conditions. The integration of bioaugmentation and biostimulation strategies has further boosted the efficiency of remediation efforts in both laboratory and field settings. In addition, innovative bioreactor designs and emerging microbial electrochemical systems illustrate promising avenues for combining remediation with energy recovery and real‐time process optimisation. Despite these advances, challenges persist, including environmental variability, regulatory constraints, economic feasibility, and ecological and biosafety concerns. Continued interdisciplinary research and collaboration are essential to overcome these obstacles, ensuring that green microbial technologies contribute effectively to sustainable environmental management and public health protection.
Keywords: Green microbial technology, bioremediation, synthetic biology, bioaugmentation, sustainability.
1. Introduction
Environmental pollution is one of the most urgent challenges of the 21st century. Rapid industrialisation, urbanisation, and unsustainable agricultural practices have led to the accumulation of hazardous substances in the air, water, and soil. Pollutants such as petroleum hydrocarbons, heavy metals, pesticides, and other xenobiotics pose significant risks to human health, ecosystems, and global biodiversity. In response, researchers and practitioners are increasingly turning to bioremediation, a process that employs living organisms to mitigate or remove environmental contaminants as a sustainable and eco‐friendly solution (Akinsemolu, Onyeaka & Arijeniwa, 2024).
Bioremediation harnesses the natural metabolic capabilities of microorganisms to transform or detoxify pollutants into less harmful substances. This approach is not only cost‐effective compared with traditional physicochemical methods but also aligns with the principles of sustainability and environmental stewardship. Advances in molecular biology, genetic engineering, and environmental monitoring have paved the way for innovative applications of microbial technology, thus enhancing the efficiency and scope of bioremediation strategies (Wu et al., 2024; Leskovac & Petrović, 2023).
The concept of “green microbial technology” represents a paradigm shift in environmental remediation. It emphasises the use of naturally occurring or engineered microorganisms that are both effective in degrading contaminants and sustainable over the long term. This approach focuses on optimising the inherent capabilities of microbial systems, often through biotechnological interventions such as genetic modification and the assembly of specialised microbial consortia (Nawaz et al., 2024; Shankar, Verma, & Shah, 2024). The aim is to maximise pollutant degradation while minimising ecological disruption and ensuring that the remediation process does not introduce new environmental challenges (Beltrán-Velasco & Clemente-Suárez, 2025).
Historically, bioremediation began with observations of indigenous microbial communities naturally degrading organic materials. Early applications were largely empirical, relying on these communities to restore environmental balance. However, as our understanding of microbial physiology and ecology deepened, it became evident that these natural processes could be harnessed and enhanced to address more complex pollutants. This realisation led to the development of targeted strategies such as bioaugmentation, the introduction of specialised microorganisms into contaminated sites and biostimulation, which involves modifying environmental conditions to enhance the activity of native degraders (Thirumalaivasan et al., 2024; Glockow et al., 2024).
The evolution of green microbial technology has been driven by the need for remediation approaches that are both effective and sustainable. Unlike traditional techniques that often rely on harsh chemicals or energy-intensive processes, microbial solutions are inherently renewable and self-sustaining. Microorganisms can adapt to diverse environmental conditions, evolve in response to pollutant stress, and even exchange beneficial genetic traits through horizontal gene transfer (Wu et al., 2024). These features enhance their degradative capabilities and contribute to the resilience of the remediation process in dynamic settings.
One of the most compelling aspects of green microbial technology is its potential to integrate with emerging innovations such as omics sciences and digital monitoring systems. Advances in metagenomics, transcriptomics, and proteomics have provided unprecedented insights into the structure and function of microbial communities in contaminated environments (Zhou et al., 2015; Zhang et al., 2021). These high-throughput techniques allow researchers to identify key microbial players, decipher metabolic pathways, and understand the complex interactions that drive pollutant degradation. When combined with real-time monitoring and sensor technologies, such insights facilitate the development of predictive models that can optimise bioremediation strategies in both laboratory and field applications (Rachbauer et al., 2024).
In recent years, the focus on green microbial technology has expanded to include the remediation of complex, mixed-contaminant systems. Industrial waste streams and urban runoff frequently contain a cocktail of pollutants that require multifaceted degradation pathways. In such scenarios, microbial consortia, diverse communities of microorganisms working synergistically show great promise (Akinsemolu et al., 2024). These consortia can be engineered or naturally selected to target multiple contaminants simultaneously, offering a more holistic approach to environmental clean-up. Moreover, synthetic biology tools further enhance this capability by enabling the design of microbial strains with improved specificity and efficiency (Nawaz et al., 2024).
Despite its promise, the application of green microbial technology is not without challenges. Variability in environmental conditions, the complexity of microbial interactions, and the potential risks associated with releasing genetically modified organisms into the environment are all significant considerations. Overcoming these challenges requires rigorous research, robust risk assessments, and transparent communication with all stakeholders (Azuazu et al., 2023). The promise of green microbial technology lies in its capacity to address environmental pollution in a manner that is both scientifically innovative and consistent with sustainable development goals.
Sustainable bioremediation offers benefits that extend beyond the immediate removal of pollutants. Effective remediation strategies can rejuvenate contaminated sites, transforming them into productive land or safe recreational areas. Furthermore, the principles underlying green microbial technology—resource efficiency, adaptability, and environmental harmony—resonate with broader societal goals of sustainability and resilience (Paniagua-Michel & Banat, 2024). By integrating green microbial technology into remediation practices, we not only achieve technical success but also reaffirm a commitment to a cleaner, healthier, and more sustainable future. This chapter is structured to provide a comprehensive exploration of the innovations and future directions in green microbial technology for sustainable bioremediation. The discussion begins with an examination of the fundamental mechanisms by which microorganisms degrade pollutants, delving into the enzymatic and metabolic pathways that underpin bioremediation and contrasting aerobic and anaerobic processes. The role of microbial genetic adaptation and physiological resilience in contaminated environments is also explored, setting the stage for harnessing and optimising these organisms for environmental clean-up.
2. Fundamentals of Microbial Bioremediation
Microbial bioremediation exploits the natural capacity of microorganisms to transform and detoxify environmental contaminants through specialised enzymatic and metabolic processes. This section provides an in-depth look at how microbes degrade pollutants, the genetic and physiological adaptations that underpin their resilience in contaminated environments, and the diverse array of microbial species that serve as the workhorses in bioremediation efforts. Where appropriate, key metabolic pathways and simplified reaction equations are provided to clarify these processes.
2.1 Microbial Degradation Mechanisms
At the core of microbial bioremediation are the enzymatic reactions that convert complex and potentially toxic compounds into simpler, less harmful substances. Microorganisms secrete a range of enzymes that catalyse specific chemical transformations. These reactions can be represented by generalised equations that illustrate the conversion of a pollutant (P) into non-toxic end products, often with the aid of cofactors such as oxygen (Wu et al., 2024).
For instance, consider the initial oxidation step mediated by monooxygenase enzymes in aerobic degradation:

In this reaction, the pollutant (P) is hydroxylated to form an alcohol derivative (P–OH), which is generally more amenable to further enzymatic attack. Subsequent steps may involve additional oxidation, cleavage, or rearrangement reactions that ultimately lead to complete mineralisation. For example, the aerobic degradation of benzene, a common environmental contaminant, proceeds via the formation of catechol as an intermediate:
2.1.1 Benzene to Phenol

2.1.2 Phenol to Catechol

Catechol then undergoes ring cleavage through either the ortho- or meta-cleavage pathway. In the ortho-cleavage pathway, catechol 1,2-dioxygenase catalyses the conversion to muconic acid, which is further metabolised into intermediates entering the tricarboxylic acid (TCA) cycle, ensuring complete degradation (Wu et al., 2024; Leskovac & Petrović, 2023).

In contrast, anaerobic degradation processes operate in environments devoid of oxygen. Here, microbes utilise alternative electron acceptors such as nitrate (NO₃⁻), sulphate (SO₄²⁻), or even carbon dioxide (CO₂) to drive metabolic reactions. For example, the anaerobic degradation of toluene can be summarised in a simplified equation when nitrate is used as the terminal electron acceptor:

Although anaerobic processes generally yield lower energy and proceed more slowly than aerobic degradation, they are critical in subsurface or sedimentary environments where oxygen is limited (Wu et al., 2024).
These degradation pathways both aerobic and anaerobic are underpinned by complex regulatory systems that sense environmental conditions and adjust enzyme expression accordingly. For instance, the presence of oxygen not only activates genes encoding oxygenases but also suppresses genes for anaerobic metabolism. Conversely, in oxygen-poor environments, regulators induce the expression of enzymes capable of utilising alternative electron acceptors.
2.3 Genetic and Physiological Adaptations
Microorganisms have evolved an array of genetic and physiological adaptations to survive and thrive in contaminated environments. These adaptations allow them to overcome the toxic effects of pollutants and even harness these compounds as energy sources. A central adaptation is the induction of stress response systems that protect cellular components from damage. Microbes upregulate detoxification enzymes such as superoxide dismutase and catalase to mitigate oxidative stress caused by reactive oxygen species generated during pollutant metabolism (Wu et al., 2024).
They also increase the production of efflux pumps, which actively expel toxic compounds from the cell. Additionally, many microbes form biofilms, a structured community of cells embedded in an extracellular polymeric substance (EPS). Biofilms provide a physical barrier against toxicants and facilitate the cooperative degradation of pollutants, as cells within biofilms can share enzymes and metabolites (Yin et al., 2019; Sharma et al., 2023).
Horizontal gene transfer (HGT) is a pivotal mechanism by which microbes acquire new capabilities, including the degradation of xenobiotics. Through processes such as conjugation, transformation, and transduction, genetic elements like plasmids and transposons can be exchanged between bacteria. For example, a plasmid carrying genes for the catabolism of hydrocarbons may be transferred from one soil bacterium to another, rapidly expanding the community’s capacity to degrade oil contaminants (Wu et al., 2024; Thirumalaivasan et al., 2024). This genetic exchange accelerates evolution, enabling microbial populations to adapt quickly to new or intensified pollutant pressures.
Mutations and natural selection also contribute to the emergence of specialised strains with enhanced bioremediation abilities. In contaminated sites, selective pressure favours microbes that can utilise pollutants as carbon or energy sources. Over time, these adaptive processes result in microbial communities that are finely tuned to the specific types and concentrations of contaminants present. A noteworthy example is the evolution of Pseudomonas putida strains with optimised pathways for the degradation of aromatic hydrocarbons, where mutations in regulatory regions lead to higher expression levels of catabolic enzymes (Leskovac & Petrović, 2023).
Physiologically, many microbes exhibit metabolic flexibility that allows them to switch between different energy-generating pathways. This flexibility is particularly important in environments where conditions such as oxygen levels or nutrient availability fluctuate. For instance, a bacterium may operate under aerobic conditions when oxygen is abundant, and shift to anaerobic metabolism during periods of oxygen limitation. Such metabolic versatility ensures that microbial communities can maintain remediation activity even as environmental conditions change (Wu et al., 2024).
2.4 Key Microbial Players
A diverse range of microorganisms including bacteria, fungi, and algae play critical roles in bioremediation. Each group offers unique enzymatic capabilities and metabolic pathways that can be harnessed to degrade specific pollutants.
Bacteria are perhaps the most extensively studied organisms in bioremediation. Genera such as Pseudomonas, Bacillus, and Acinetobacter are well-known for their ability to degrade hydrocarbons, pesticides, and other organic compounds. For example, Pseudomonas species are frequently utilised in the bioremediation of oil spills due to their ability to produce oxygenases that catalyse the initial oxidation of hydrocarbons. Their metabolic pathways not only break down complex compounds into simpler molecules but also channel these intermediates into central metabolic cycles, ensuring complete mineralisation (Henriksen, 2023).
Fungi contribute significantly to bioremediation, especially in the degradation of recalcitrant pollutants that resist bacterial attack. Mycoremediation leverages the enzymatic prowess of fungi, such as white-rot fungi like Phanerochaete chrysosporium. These fungi secrete lignin peroxidase, manganese peroxidase, and laccase, enzymes capable of degrading complex aromatic structures, including polycyclic aromatic hydrocarbons (PAHs) and synthetic dyes. The filamentous structure of fungi allows them to penetrate soil matrices, accessing pollutants that are otherwise shielded from bacterial degradation. Their ability to degrade lignin, a complex natural polymer, is analogous to breaking down similarly structured synthetic compounds (Thirumalaivasan et al., 2024).
Algae are particularly useful in the remediation of aquatic environments. Certain algal species possess the ability to bioaccumulate heavy metals and nutrients from water bodies, effectively reducing concentrations of these toxic elements. For instance, algae such as Chlorella and Scenedesmus can adsorb heavy metals like cadmium, lead, and mercury, incorporating them into their biomass; a process termed biosorption. Moreover, when used in tandem with bacterial communities, algae can enhance the overall degradation of contaminants by supplying oxygen through photosynthesis, thereby stimulating aerobic degradation processes in the surrounding environment (Glockow et al., 2024).
A practical illustration of these microbial players working in concert can be seen in integrated remediation systems. In an oil-contaminated soil scenario, a consortium might be developed in which Pseudomonas initiates the breakdown of aliphatic and aromatic hydrocarbons, while Phanerochaete chrysosporium degrades more complex residual compounds. In a parallel aquatic system, algae could sequester heavy metals, reducing toxicity and promoting the growth of pollutant-degrading bacteria. These case examples highlight the synergistic effects of employing multiple microbial groups, where the combined metabolic capabilities lead to a more comprehensive remediation process (Paniagua-Michel & Banat, 2024; Henriksen, 2023).
The effectiveness of these key microbial players is further enhanced when they are supported by modern biotechnological interventions. Genetic engineering and synthetic biology allow for the optimisation of natural pathways and the construction of microbial consortia with tailored degradative profiles. By identifying and overexpressing specific genes encoding for critical enzymes—such as oxygenases, dehydrogenases, and peroxidases, researchers can significantly boost the efficiency of pollutant degradation. Moreover, engineered pathways can be designed to minimise the production of toxic intermediates, ensuring that the remediation process is both effective and environmentally benign (Akinsemolu et al., 2024).
The fundamentals of microbial bioremediation rest on the ability of microorganisms to deploy a diverse arsenal of enzymatic reactions to convert pollutants into harmless end products. The detailed enzymatic pathways, whether under aerobic or anaerobic conditions, involve well-orchestrated sequences of oxidation, reduction, and ring-cleavage reactions. Coupled with robust genetic and physiological adaptations, ranging from stress responses and biofilm formation to horizontal gene transfer and metabolic flexibility, these microbial systems are uniquely equipped to tackle environmental contaminants. The collaborative actions of bacteria, fungi, and algae further expand the scope and effectiveness of bioremediation strategies, making green microbial technology a cornerstone of sustainable environmental remediation. Through continuous research and innovation, these processes are being refined to offer even more efficient, scalable, and ecologically sound solutions for the challenges posed by industrial and urban pollution (Glockow et al., 2024; Jalali et al., 2023).
3. Advances in Green Microbial Technologies
Advances in green microbial technologies have ushered in a new era of sustainable environmental remediation. Recent innovations, from genetic engineering to the integration of digital monitoring tools, are transforming our ability to harness and enhance microbial processes for pollutant degradation. This section examines several key areas of progress that are contributing to more efficient and resilient bioremediation strategies.
3.1 Genetic Engineering and Synthetic Biology
The advent of genetic engineering and synthetic biology has revolutionised the field of microbial bioremediation. Using cutting-edge tools such as CRISPR and other gene-editing techniques, researchers can precisely modify microbial genomes to enhance their natural degradative abilities. These methods enable the overexpression of enzymes that catalyse key steps in pollutant breakdown, thus accelerating metabolic pathways that would otherwise proceed at a suboptimal rate (Nawaz et al., 2024).
Beyond the enhancement of individual strains, genetic engineering facilitates the construction of synthetic microbial consortia with complementary metabolic capabilities. By designing these custom communities, researchers can combine microbes possessing diverse catabolic pathways to create a synergistic system better equipped to tackle complex contaminant mixtures. This approach not only improves degradation performance but also enhances resilience under fluctuating environmental conditions. Moreover, synthetic biology extends to the design of novel regulatory circuits that can be programmed to respond dynamically to the presence of specific pollutants, activating degradation pathways only when required. Such inducible systems minimise the metabolic burden on microbes during periods of low contamination and improve overall system efficiency (Nawaz et al., 2024).
3.2 Bioaugmentation and Biostimulation Strategies
Bioaugmentation and biostimulation are two complementary strategies that play a crucial role in enhancing microbial bioremediation. Bioaugmentation involves the deliberate introduction of specialised microorganisms into contaminated sites. These exogenous strains, selected for their proven degradative capabilities, work alongside indigenous microbial communities to kick-start the remediation process in environments where native microbial activity is insufficient (Henriksen, 2023).
In contrast, biostimulation focuses on optimising in situ conditions to encourage the growth and activity of indigenous microbial populations. This strategy involves amending contaminated sites with nutrients, electron donors, or electron acceptors to create an environment conducive to the metabolic processes required for pollutant degradation. For example, the addition of organic substrates can stimulate heterotrophic bacteria capable of breaking down complex organic compounds, while introducing nitrate or sulphate can enhance anaerobic processes in oxygen-limited areas. Both strategies demand a detailed understanding of the local microbial ecology and site-specific physicochemical conditions, and they often work best when combined as part of a holistic remediation approach (Henriksen, 2023).
3.3 Novel Bioreactor Designs and In Situ Applications
Controlled remediation in bioreactors represents another significant advancement in green microbial technologies. Bioreactors provide a controlled environment where factors such as temperature, pH, oxygen concentration, and nutrient levels can be precisely managed to maximise microbial activity. This approach is particularly beneficial for treating industrial effluents or highly concentrated waste streams, where the kinetics of microbial degradation can be closely monitored and optimised (Rachbauer et al., 2024).
Several bioreactor configurations have been developed to suit varying contaminant types and operational requirements. Fluidised bed reactors, for instance, promote intimate contact between microbial biomass and pollutants by suspending the cells in a continuously moving fluid, while packed-bed reactors utilise immobilised microbial cells on a solid support, offering high cell densities and prolonged contact times. Each configuration is selected based on the nature of the pollutant and the specific remediation goals, highlighting the versatility of bioreactor designs (Finny, 2024).
Field applications of microbial bioremediation typically fall into two broad categories: in situ and ex situ systems. In situ bioremediation treats contaminants directly at the site, minimising excavation and transport, and is generally less disruptive and more cost-effective for large or diffuse contamination plumes. Ex situ bioremediation, however, involves the removal of contaminated material to a treatment facility where conditions can be strictly controlled in a bioreactor setting. Although ex situ methods generally incur higher costs and logistical challenges, they often achieve faster remediation rates when on-site conditions are too variable or extreme for effective in situ treatment. Increasingly, hybrid solutions are emerging that combine elements of both approaches to enhance flexibility and overall remediation efficiency (Rachbauer et al., 2024).
3.4 Integration with Digital Technologies
The integration of digital technologies into green microbial remediation practices is rapidly transforming the way in which bioremediation processes are monitored, controlled, and optimised. Biosensors, for example, have become invaluable tools for the real-time monitoring of key parameters such as pollutant concentration, microbial activity, and environmental conditions. These sensors can be deployed in both laboratory bioreactors and field sites, providing continuous data streams that enable dynamic adjustments during the remediation process (Rachbauer et al., 2024).
Advanced omics technologies such as metagenomics and transcriptomics are increasingly being applied to decipher the complex microbial community dynamics at contaminated sites. Metagenomics allows for the comprehensive analysis of all genetic material present, revealing the diversity of microbial species and the functional genes they harbour. This information is crucial for identifying active degradation pathways and pinpointing potential bottlenecks in the remediation process. Meanwhile, transcriptomics provides insights into gene expression profiles under specific environmental conditions, offering a real-time snapshot of microbial metabolic activity. The integration of data from these techniques into predictive models and machine-learning algorithms enables the optimisation of operational parameters and the anticipation of remediation outcomes (Shyam et al., 2023).
Table 1 summarises the key technological advances in green microbial remediation, highlighting the main features, applications, advantages, and limitations associated with each approach.
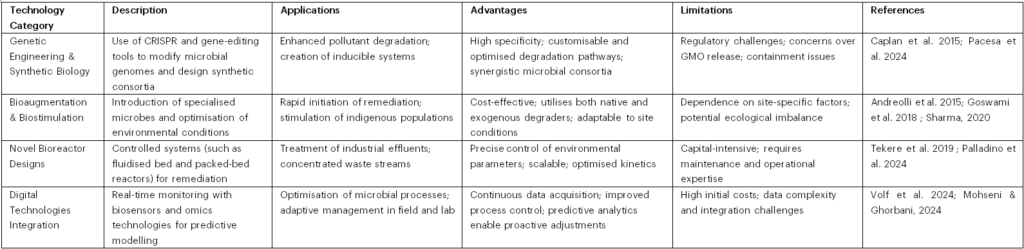
6. Conclusion
The findings of the study affirm that Business Education significantly contributes to national development despite facing numerous challenges. Strategies to mitigate insecurity in Nigeria include providing proper laboratories for Business Education to facilitate skill acquisition, enhancing students’ attitudes towards skill learning through orientation, regularly monitoring Business Education programs to ensure quality, and adequately funding these programs.
5. Case Studies and Innovations
This section explores real-world examples and cutting-edge developments in the field of microbial bioremediation. It illustrates how green microbial technologies are being applied in diverse contexts—from oil spill cleanup operations to heavy metal immobilisation—and highlights emerging innovations that promise to further enhance the sustainability and efficiency of remediation efforts.
5.1 Petroleum Hydrocarbon Remediation
Petroleum hydrocarbon contamination is one of the most extensively studied areas in bioremediation, owing to the environmental and economic impacts of oil spills and industrial discharges. Numerous case studies from around the world provide detailed insights into both laboratory research and large-scale field applications of microbial remediation techniques.
In the wake of major oil spills, such as the Deepwater Horizon incident in the Gulf of Mexico, indigenous microbial communities were observed to respond rapidly to the influx of hydrocarbons. Field studies revealed that certain strains, particularly those belonging to the genus Pseudomonas, were capable of metabolising a wide range of aliphatic and aromatic hydrocarbons. Laboratory experiments have further refined our understanding of these processes by characterising the enzymatic pathways involved. For instance, researchers have demonstrated that oxygenases catalyse the initial oxidation of hydrocarbons, converting them into more hydrophilic compounds that can be more readily assimilated into central metabolic pathways (Henriksen, 2023; Paniagua-Michel & Banat, 2024).
One notable example comes from a controlled bioreactor study, where artificially contaminated soil was treated using a bioaugmentation approach. Specific Pseudomonas strains, isolated for their hydrocarbon-degrading capacity, were introduced into the soil along with a nutrient amendment to stimulate the indigenous microbial population. The results showed a significant reduction in total petroleum hydrocarbons (TPH) over a period of weeks, with the degradation kinetics closely monitored through gas chromatography–mass spectrometry (GC-MS). These laboratory findings have been successfully translated into field-scale applications, where combined bioaugmentation and biostimulation strategies have led to effective remediation of contaminated shorelines and coastal sediments (Henriksen, 2023; Paniagua-Michel & Banat , 2024).
The integration of digital monitoring tools in these case studies has further enhanced the operational efficiency of petroleum hydrocarbon remediation. Biosensors and real-time data acquisition systems are used to monitor oxygen levels, nutrient concentrations, and microbial activity, allowing for dynamic adjustments during the remediation process. Such technologies have improved the predictability and control of bioremediation outcomes, making them an integral part of modern oil spill response strategies (Rachbauer et al., 2024).
5.2 Heavy Metal and Metalloids Bioremediation
Heavy metal and metalloid contamination presents a different set of challenges compared to organic pollutants, as these elements are non-biodegradable and persist in the environment. However, microbes can play a crucial role in transforming and immobilising these toxic substances, thereby reducing their bioavailability and ecological impact.
Microbial bioremediation of heavy metals often relies on mechanisms such as biosorption, bioaccumulation, and enzymatic reduction. Certain bacteria and fungi have the capacity to alter the oxidation state of metals, rendering them less toxic. For example, bacteria in the genus Bacillus have been reported to reduce hexavalent chromium (Cr (VI)) to the less toxic trivalent form (Cr (III)) through reductive pathways. Similarly, fungi such as Aspergillus niger are capable of accumulating heavy metals within their biomass via biosorption, a process that is mediated by functional groups on the cell wall (Cozma et al., 2024).
Several case studies highlight the successful application of these mechanisms in contaminated environments. In one study conducted at a former mining site, researchers introduced a consortium of metal-tolerant bacteria into the soil. Over a period of several months, the consortium facilitated the conversion of soluble lead and cadmium into insoluble forms, effectively immobilising the metals and reducing leachate into the surrounding water bodies. Detailed chemical analyses confirmed that the transformation processes were mediated by microbial enzymes and cell surface interactions, which promoted the formation of stable metal complexes (Magrini et al., 2025; Cao et al., 2025).
Despite these successes, the bioremediation of heavy metals remains challenging due to the potential for re-mobilisation under changing environmental conditions. Case studies often report the necessity of maintaining specific pH and redox conditions to sustain the immobilised state of the metals. Moreover, the long-term stability of the biogenic precipitates is a critical factor in ensuring that remediation efforts yield lasting environmental benefits. Researchers continue to investigate the role of microbial communities in sustaining these conditions, with a focus on the genetic regulation of metal reduction pathways and the optimisation of biofilm formation, which can act as a natural stabiliser in contaminated sites (Cozma et al., 2024).
5.3 Emerging Technologies
While traditional bioremediation approaches have demonstrated considerable success, emerging technologies are pushing the boundaries of what is possible by integrating microbial processes with innovative engineering and digital solutions as seen in Figure 1.
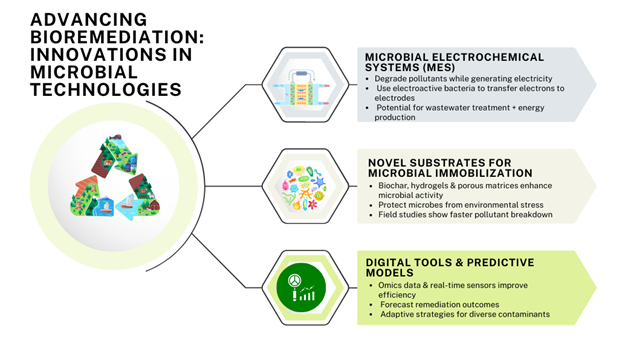
Figure 1: Advancing Bioremediation Innovations in Microbial Technologies
One promising area is the development of microbial electrochemical systems (MES). MES not only facilitate the degradation of pollutants but also enable the recovery of energy in the form of electricity. In these systems, specialised electroactive bacteria transfer electrons to an electrode during the oxidation of organic contaminants. This process, known as extracellular electron transfer, effectively couples bioremediation with energy recovery. Laboratory-scale MES have shown the potential to treat wastewater while generating measurable electrical outputs, paving the way for dual-purpose treatment facilities that combine remediation with sustainable energy production (Rachbauer et al., 2024).
Another emerging technology involves the use of novel substrates and carriers for immobilising microbes in contaminated sites. Advances in material science have led to the development of biochar, hydrogels, and other porous matrices that provide a stable habitat for microbial communities. These materials enhance the contact between microbes and pollutants, while also protecting the microorganisms from environmental stresses. For instance, a recent field study employed biochar-amended soils to support the growth of hydrocarbon-degrading bacteria, resulting in a significant acceleration of pollutant breakdown compared to unamended soils. The novel carriers not only improve degradation rates but also facilitate the recovery and reuse of microbial biomass, contributing to the overall sustainability of the remediation process (Finny, 2024).
Emerging technologies also benefit from the integration of digital and computational tools. The use of advanced modelling techniques, informed by omics data and real-time sensor readings, is leading to the development of predictive frameworks that can forecast remediation outcomes. These models incorporate variables such as pollutant concentration, microbial community dynamics, and environmental conditions, enabling practitioners to design and implement more effective bioremediation strategies. The convergence of traditional microbiology with digital innovations is creating a versatile toolkit that is adaptable to a wide range of contaminants and environmental settings (Mohseni & Ghorbani, 2024).
In summary, case studies and innovations in microbial bioremediation illustrate how advances in technology are being applied to real-world problems. From the dynamic responses observed in petroleum hydrocarbon remediation to the specialised mechanisms involved in heavy metal transformation, and the promising new avenues offered by microbial electrochemical systems and novel substrates, these examples highlight the potential of green microbial technologies. As research continues to integrate digital tools with traditional bioremediation methods, the field is poised to deliver even more efficient, sustainable, and scalable solutions for environmental restoration (Bikram Jit Singh et al., 2023).
6. Challenges and Limitations
While advances in green microbial technologies have significantly expanded the potential for sustainable environmental remediation, several challenges and limitations remain that must be addressed to ensure the effective and safe implementation of these approaches. These challenges span environmental, operational, regulatory, economic, social, and ecological dimensions as shown in Figure 2.
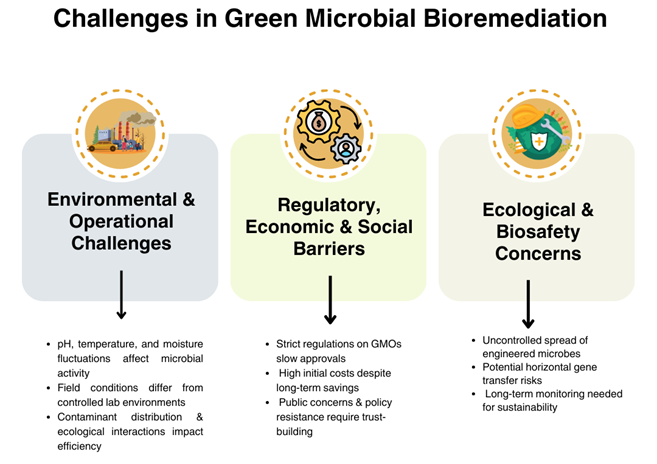
Figure 2: Challenges in Green Microbial Bioremediation
6.1 Environmental and Operational Factors
One of the foremost challenges in microbial bioremediation is the inherent variability of environmental conditions. Factors such as pH, temperature, moisture, and the presence of other chemical contaminants can profoundly influence microbial activity. For example, deviations in pH can alter enzyme structure and function, while temperature fluctuations may affect metabolic rates and microbial growth. Moisture levels are critical in soil and sediment environments, as insufficient water can limit the diffusion of nutrients and contaminants, thereby reducing microbial efficacy (Bikram Jit Singh et al., 2023).
Furthermore, laboratory conditions are typically highly controlled and optimised to support microbial activity and pollutant degradation. However, scaling these successes to field conditions often presents significant obstacles. In the field, heterogeneous distributions of contaminants and variable environmental parameters can lead to inconsistent performance of microbial treatments. Additionally, the complexity of natural ecosystems introduces competition, predation, and other ecological interactions that are not typically encountered in laboratory settings. These factors can compromise the efficiency of bioremediation processes and necessitate the development of more robust and adaptable strategies (Bikram Jit Singh et al., 2023).
6.3 Regulatory, Economic, and Social Considerations
Beyond the technical challenges, the commercialisation of green microbial technologies faces a number of regulatory, economic, and social hurdles. Regulatory frameworks governing the release and use of genetically modified organisms (GMOs) are particularly stringent, given the potential risks associated with their uncontrolled spread in open environments. Securing approval for field applications often requires extensive risk assessments and compliance with national and international guidelines, which can delay implementation and increase costs (Azuazu et al., 2023).
Economic feasibility is another critical consideration. Although bioremediation is often more cost-effective in the long term compared to conventional physicochemical methods, the initial investments required for research, development, and scaling-up can be substantial. Detailed cost–benefit analyses are essential to evaluate the economic viability of these technologies, particularly in comparison with established remediation methods. Moreover, securing funding for large-scale projects can be challenging, particularly in regions where financial resources are limited.
Social acceptance is also vital for the successful deployment of bioremediation projects. Public concerns regarding the use of GMOs and uncertainties about the long-term effects of microbial interventions can influence policy decisions and community support. Transparent communication, stakeholder engagement, and robust risk management strategies are necessary to address these concerns and foster trust among local communities and regulatory bodies (Akinsemolu, 2023)
6.4 Ecological and Biosafety Concerns
The ecological implications of introducing engineered microorganisms into open environments remain a significant area of concern. One of the primary biosafety issues is the risk associated with the release of genetically modified organisms (GMOs). Once released, it is challenging to control the spread and persistence of these organisms, which could lead to unintended consequences for local ecosystems. The horizontal gene transfer between introduced strains and native microbes is another potential risk, as it may result in the dissemination of engineered traits beyond their intended targets (Thirumalaivasan et al., 2024).
Long-term ecological impacts are also a critical consideration. While microbial interventions are designed to be sustainable and environmentally benign, the dynamic nature of ecosystems means that the outcomes of such interventions may change over time. Continuous monitoring is required to ensure that microbial activity does not lead to adverse effects, such as the accumulation of toxic intermediates or the disruption of native microbial communities. Additionally, the sustainability of these interventions depends on their ability to maintain long-term efficacy without necessitating repeated applications, which could increase the ecological footprint of the remediation process (Bikram Jit Singh et al., 2023).
In conclusion, while green microbial technologies offer promising and sustainable solutions for environmental remediation, overcoming these challenges is essential to ensure their safe, effective, and economically viable implementation. Addressing environmental variability, scaling laboratory successes to the field, navigating regulatory and economic barriers, and mitigating ecological risks will require continued interdisciplinary research and collaborative efforts among scientists, policymakers, industry stakeholders, and local communities.
7. Conclusion
Green microbial technologies offer a transformative approach to environmental remediation by harnessing the natural abilities of microorganisms to detoxify contaminated ecosystems. This chapter has outlined the fundamental microbial degradation mechanisms—both aerobic and anaerobic—and demonstrated how genetic and physiological adaptations underpin effective pollutant breakdown. Advances in genetic engineering and synthetic biology have enabled the customisation of microbial strains and consortia, while bioaugmentation and biostimulation enhance the activity of both introduced and indigenous microbes. Innovative bioreactor designs and in situ applications have successfully translated laboratory findings to field-scale operations, and the integration of digital technologies has improved real-time monitoring and process optimisation. However, challenges remain in adapting these technologies to variable environmental conditions, navigating regulatory and economic hurdles, and addressing ecological and biosafety concerns. In essence, while obstacles persist, the convergence of scientific innovation, technological integration, and practical application underscores the potential of green microbial technologies to advance sustainable environmental remediation. Continued interdisciplinary research and collaboration will be key to overcoming these challenges and ensuring that these approaches contribute effectively to the restoration of polluted environments.
Conflict of Interest
The author declares no conflict of interest.
References
Akinsemolu, A. A. (2023). Principles of green microbiology: The microbial blueprint for sustainable development. Environmental Advances, 14, 100440. https:// doi.org/10.1016/j.envadv.2023.100440
Akinsemolu, A. A., Onyeaka, H., & Arijeniwa, F. V. (2024). Harnessing green microbiology for sustainable water management, agriculture, and energy generation: Current advances and future prospects. World Water Policy, 10(3), 649-697. https://doi.org/10.1002/wwp2.1221
Azuazu, I. N., Sam, K., Campo, P., & Coulon, F. (2023). Challenges and opportunities for low-carbon remediation in the Niger Delta: Towards sustainable environmental management. Science of the Total Environment, 900, 165739. https://doi.org/10.1016/j.scitotenv.2023.165739
Beltrán-Velasco, A. I., & Clemente-Suárez, V. J. (2025). Harnessing gut microbiota for biomimetic innovations in health and biotechnology. Biomimetics, 10(2), 73. https://doi.org/10.3390/biomimetics10020073
Bikram Jit Singh, A., Chakraborty, A., & Sehgal, R. (2023). A systematic review of industrial wastewater management: Evaluating challenges and enablers. Journal of Environmental Management, 348, 119230. https://doi.org/10.1016/j.jenv man.2023.119230
Cao, X., Dong, Q., Mao, L., Yang, X., Wang, X., & Zou, Q. (2025). Enhanced phytoextraction technologies for the sustainable remediation of cadmium‐contaminated soil based on hyperaccumulators—A review. Plants, 14, 115–115. https://doi.org/10.3390/plants14010115
Cozma, P., Bețianu, C., Hlihor, R. M., Simion, I. M., & Gavrilescu, M. (2024). Bio‐recovery of metals through biomining within circularity‐based solutions. Processes, 12, 1793–1793. https://doi.org/10.3390/pr12091793
Finny, A. (2024). 3D bioprinting in bioremediation: A comprehensive review of principles, applications, and future directions. PeerJ, 12. Retrieved from https://www.semanticscholar.org/paper/49ecc56ca7444668f3657b6e2ce72ad6bc1e56ff
Glockow, T., Kaster, A. K., & Rabe, K. S. (2024). Sustainable agriculture: Leveraging microorganisms for a circular economy. Applied Microbiology and Biotechnology, 108, 452. https://doi.org/10.1007/s00253-024-13294-0
Henriksen, M. (2023). Bioremediation of hydrocarbon contaminated soil: Exploring the viability for contaminated grounds and landfills in Norway (Master’s thesis, University of Stavanger).
Jalali, F. M., Chahkandi, B., Gheibi, M., Eftekhari, M., Behzadian, K., & Campos, L. C. (2023). Developing a smart and clean technology for bioremediation of antibiotic contamination in arable lands. Sustainable Chemistry and Pharmacy, 33, 101127. https://doi.org/10.1016/j.scp.2023.101127
Leskovac, A., & Petrović, S. (2023). Pesticide use and degradation strategies: Food safety, challenges and perspectives. Foods, 12, 2709–2709. https://doi.org/10. 3390/foods12142709
Magrini, C., Verga, F., Bassani, I., Pirri, C. F., & Abdel Azim, A. (2025). A microbial‐centric view of mobile phones: Enhancing the technological feasibility of biotechnological recovery of critical metals. Bioengineering, 12, 101–101. https://doi.org/10.3390/bioengineering12020101
Mohseni, P., & Ghorbani, A. (2024). Exploring the Synergy of Artificial Intelligence in Microbiology: Advancements, Challenges, and Future Prospects. Computational and Structural Biotechnology Reports, 100005. https://doi.org/10.1016 /j.csbr.2024.100005
Nawaz, T., Gu, L., Hu, Z., Fahad, S., Saud, S., & Zhou, R. (2024). Advancements in synthetic biology for enhancing cyanobacterial capabilities in sustainable plastic production: A green horizon perspective. Fuels, 5, 394–438. https://doi.org/10.3390/fuels5030023
Pacesa, M., Pelea, O., & Jinek, M. (2024). Past, present, and future of CRISPR genome editing technologies. Cell, 187(5), 1076-1100. https://www.cell.com /cell/fulltext/S0092-8674(24)00111-9
Paniagua-Michel, J., & Banat, I. M. (2024). Unravelling diatoms’ potential for the bioremediation of oil hydrocarbons in marine environments. Clean Technologies, 6(1), 93-115. https://doi.org/10.3390/cleantechnol6010007
Rachbauer, L., Granda, C. B., Shrestha, S., Fuchs, W., Gabauer, W., Singer, S. W., Simmons, B. A., & Urgun-Demirtas, M. (2024). Energy and nutrient recovery from municipal and industrial waste and wastewater—a perspective. Journal of Industrial Microbiology and Biotechnology, 51, kuae040. https://doi.org /10.1093/jimb/kuae040
Shankar, J., Verma, P., & Shah, M. P. (Eds.). (2024). Microbial approaches for sustainable green technologies. CRC Press.
Sharma, S., Mohler, J., Mahajan, S. D., Schwartz, S. A., Bruggemann, L., & Aalinkeel, R. (2023). Microbial biofilm: a review on formation, infection, antibiotic resistance, control measures, and innovative treatment. Microorganisms, 11(6), 1614. https://doi.org/10.3390/microorganisms11061614
Shyam, K., Kumar, N., Chandel, H., Dogra, A. S., Sharma, G., & Saxena, G. (2023). Omics Technologies in Environmental Microbiology and Microbial Ecology: Insightful Applications in Bioremediation Research. Genomics Approach to Bioremediation: Principles, Tools, and Emerging Technologies, 433-454. https://doi.org/10.1002/9781119852131.ch23
Thirumalaivasan, N., Gnanasekaran, L., Kumar, S., Durvasulu, R., Sundaram, T., Rajendran, S., Nangan, S., & Kanagaraj, K. (2024). Utilization of fungal and bacterial bioremediation techniques for the treatment of toxic waste and biowaste. Frontiers in Materials, 11, 1416445. https://doi.org/10.3389/fmats .2024.1416445
Wu, J., Peng, H., Cheng, P., Liu, H., Zhang, Y., & Gong, M. (2024). Microbial degradation mechanisms, degradation pathways, and genetic engineering for pyrethroids: Current knowledge and future perspectives. Critical Reviews in Toxicology, 1–25. https://doi.org/10.1080/10408444.2024.2433632
Yin, W., Wang, Y., Liu, L., & He, J. (2019). Biofilms: the microbial “protective clothing” in extreme environments. International journal of molecular sciences, 20(14), 3423. https://doi.org/10.3390/ijms20143423
Zhang, L., Chen, F., Zeng, Z., Xu, M., Sun, F., Yang, L., … & Xie, Y. (2021). Advances in metagenomics and its application in environmental microorganisms. Frontiers in microbiology, 12, 766364. https://doi.org/10.3389/fmicb.2021.766364
Zhou, J., He, Z., Yang, Y., Deng, Y., Tringe, S. G., & Alvarez-Cohen, L. (2015). High-throughput metagenomic technologies for complex microbial community analysis: open and closed formats. MBio, 6(1), 10-1128. https://doi.org/10. 1128/mBio.02288-14
About this Article
Cite this Article
APA
Akinsemolu A.A. (2025).Harnessing Green Microbial Technology for Sustainable Bioremediation: Innovations and Future Directions. In Akinyele B.J., Kayode R. & Akinsemolu A.A. (Eds.), Microbes, Mentorship, and Beyond: A Festschrift in Honour of Professor F.A. Akinyosoye. SustainE
Chicago
Akinsemolu A.A. 2025. “Harnessing Green Microbial Technology for Sustainable Bioremediation: Innovations and Future Directions” In Microbes, Mentorship, and Beyond: A Festschrift in Honour of Professor F.A. Akinyosoye, edited by Akinyele B.J., Kayode R. and Akinsemolu A.A., SustainE.
Received
15 December 2024
Accepted
10 January 2025
Published
4 February 2025
Corresponding Author Email: akinsemoluaa@aceondo.edu.ng
Disclaimer: The opinions and statements expressed in this article are the authors’ sole responsibility and do not necessarily reflect the viewpoints of their affiliated organizations, the publisher, the hosted journal, the editors, or the reviewers. Furthermore, any product evaluated in this article or claims made by its manufacturer are not guaranteed or endorsed by the publisher.
Distributed under Creative Commons CC-BY 4.0
Share this article
Use the buttons below to share the article on desired platforms.