Adeleke, Demilade Folasade 1 Gabriel-Ajobiewe, Ruth Adefolakemi O. 1
1Department of Microbiology, Federal University Oye-Ekiti, Ekiti-State, Nigeriabr> *Corresponding Author Email:
Abstract
Saccharomyces cerevisiae has been utilised by industries over the years for ethanol production. Needless to say, it is a ‘must-have’ starter in the winemaking industry. However, over-accumulation of ethanol has been recorded to have a toxic effect on Saccharomyces cerevisiae. Consequently, there is a limit to the level of ethanol production achieved by this industrially relevant yeast, which is detrimental to the industrial setting. Therefore, This study aims to engineer Saccharomyces cerevisiae through Adaptive Laboratory Evolution (ALE) to alleviate the menace of limited ethanol tolerance in Saccharomyces cerevisiae. Wild strain Saccharomyces cerevisiae characterisedfrom previous research was obtained and purified. An ethanol tolerance test was carried out to ascertain the initial tolerance level of the wild strain before ALE. ALE was carried out in 12 generations, gradually increasing ethanol concentration with each successive generation.The wild isolate and the adapted isolates from cycle 7a (36 hours), 7b (72 hours), 10a (36 hours) and 10b (72 hours) were subjected to expressional studies using q-PCR. Four (4) genes identified to be involved in ethanol tolerance were targeted: PCK1, LSC1, ADH2, and ACS1. An internal control gene (ACT1) was also adopted. The wild isolate had an initial ethanol tolerance level of 7.0%, which increased gradually during evolution and peaked at 11.5%. At 12% and 12.5%, the optical density of the isolates began to decline.
Keywords: Adaptive Laboratory Evolution, Ethanol Tolerance, Gene Expression.
1. Introduction
Adaptive Laboratory Evolution (ALE) is a method in biological studies that helps to gain insights into the basic mechanisms of molecular evolution and adaptive changes that accumulate in microbial populations during long-term selection under specified growth conditions (Liu et al., 2015). ALE has been used extensively to rationally enhance the functionality of the microbial cell factories and boost their secretion capacity (Mattenberger et.al., 2021). This has been successfully demonstrated for chemical production (Xu et al., 2018) and enzyme production (Packer & Liu, 2015) used in pharmaceuticals (Ottilie et al., 2022) and other bioprocesses. No doubt, experimental evolution has proved, with its numerous benefits, to be a choice method for studying evolutionary processes (Dettman et al., 2012). Some studies have shown that it is mostly preferred to classical genetic engineering but also has some inherent limitations. However, recent studies show how some of the limitations may be overcome to successfully incorporate ALE in microbial cell factory design (Gresham & Dunham, 2014).
Evolution experiments often focus on changing nutrient availability, culturing conditions, and studying an organism’s evolutionary response to substances or stress conditions (Packer & Liu, 2015; Good et al., 2017). Microbes are mostly preferred for such experiments due to their short generation times, large population sizes, and Simple nutrient requirements (Troy et al., 2019; Dettman et al., 2012). This approach has been successfully used in several microbes (Choe et al., 2019), including the eukaryote S. cerevisiae (Araya et al., 2010; Chang et al., 2013).
The ability to convert sugar to ethanol is a major trait of Saccharomyces cerevisiae, making it desirableto the industrial community (Chiranjeevi et al., 2013). Coupled with this is its ability to use alternative carbon sources by adapting growth profiles (Djegui et al., 2014; Smets et al., 2010). This microbial cell factory has been used for several biotechnological tasks, including biofuel production and the production of other industrial and biopharmaceutical products (Shendure et al., 2017). That said, due to the inimical effects of ethanol over-accumulation on Saccharomyces cerevisiae, there is a limit to the level of ethanol production achievable by it. However, this can be salvaged by harnessing new expertise and biotechnological skills to exploit the yeast’s industrial potential better. Undoubtedly, this industrially relevant yeast can achieve so much more with increased ethanol tolerance. Hence, Adaptive Laboratory Evolution (ALE) was utilised in this study to generate a more potentially robust and optimised microbial system in Saccharomyces cerevisiae. The main aim of this study is to exploit the microbial cell factory of S. cerevisiae, with a view to increasing its ethanol tolerance via system engineering and to determine the effect of ALE on the expressional level of some selected genes relating to ethanol tolerance in S. cerevisiae.
2. Research Methodology
2.1 Purification of Saccharomyces cerevisiae
Wild-typeSaccharomyces cerevisiae was obtained from previous research and purified using Yeast Extract Potato Dextrose Agar (YEPD).
2.2 Initial ethanol tolerance of Saccharomyces cerevisiae
The initial ethanol tolerance ofSaccharomyces cerevisiae was tested by culturing Saccharomyces cerevisiae on YEPD broth at different ethanol concentrations. A total of 21 concentrations were used. The lowest was 0.5g/l, from which all other concentrations varied with an increase of 0.5g/l. The highest tested concentration was 10.5 g/l (Stanley et al., 2010; LaCroix et al., 2017).
2.3 Adaptive Laboratory Evolution
Saccharomyces cerevisiae was subjected to ethanol-induced stress using serial batch culture, gradually increasing the ethanol concentration by 0.5% with each generation across 12 generations. Each generation was cultured in 2 successive cycles to allow for adaptation, summing up to 24 cycles. The optical density of each culture was examined with a spectrophotometer at 0 hour, 36 hours, and 72 hours. ALE was carried out in 5 replicates, each in a 100ml conical flask. Each cycle had a control, and at the end of each cycle, the replicate with the highest optical density was subcultured to begin the next cycle (Avrahami-Moyal et al., 2012; Xu et al., 2018).
2.4 Extraction and q-PCR of wild and adapted strain
RNA was extracted from wild Saccharomyces cerevisiae (cycle 0) and adapted Saccharomyces cerevisiae isolated from cycle 7a, cycle 7b, cycle 10a and 10b. 4 genes (PCK1, LSC1, ADH2 and ACS1) identified in the literature to be associated with ethanol tolerance in Saccharomyces cerevisiae were selected. An internal control gene –ACT1 was also selected. Gene primers were designed using gene runner and synthesised, and the selected strains were subjected to q-PCR to study the expression of said genes in the wild type as against the adapted strains selected from different generations of ALE (Thornton & Basu, 2011).
2.5 Preparation of Standard Liquid Media for the Growth of Fungi and Bacteria
Thirteen 13 g of nutrient broth powder was dissolved in 1 L of water, while 30 g of Sabouraud dextrose agar was dissolved into 1 L of water as standard liquid media for culturing bacteria and fungi, respectively. They were dispensed into smaller conicals, and the resulting media were sterilized at 121 C for 15 min. Media were allowed to cool after sterilization before inoculation with the selected microbe. For the standard solidified media, 28 g of Nutrient agar and 65 g of Sabouraud dextrose agar were dissolved in 1 L of water, sterilized and allowed to cool, respectively.
3. Results
3.1 Initial Ethanol Tolerance
Figure 1 shows the yeast’s initial ethanol tolerance before adaptation. After a 72-hour culture period on yeast extract potato dextrose agar and broth, the isolated wild S. cerevisiae showed visible growth at 0.5g/l, 1.0g/l, 1.5g/l… and 7.0g/l of ethanol. At 7.5g/l—11g/ll, there was no visible growth, indicating that at 7.5g/l, ethanol had a tidal effect on this wild strain of S. cerevisiae with tolerance of below 7.5g/l.
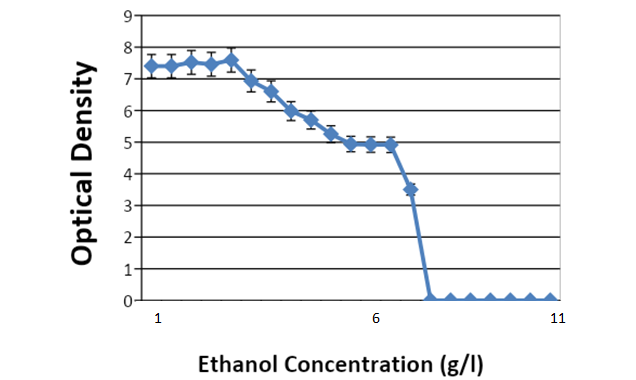
Figure 1: Initial Ethanol Tolerance Test for Saccharomyces cerevisiae
The line graph represents the mean value of the entire ethanol concentration in each test tube (n=3). The mean ethanol tolerance is not statistically significant at p<0.05.
3.2 Adaptive Laboratory Evolution
Figure 2 shows the mean values spectrophotometric readings taken at intervals during the adaptation of S. cerevisiae for increased ethanol tolerance. From 7.0g/l (the highest threshold of ethanol tolerance for the wild strain) to 10.5g/l, a steady increase in microbial load was recorded. This shows that in terms of adaptation/domestication, the test strain could survive and thrive at higher levels of ethanol. At 11.5g/l and 12g/l, there was no increase in microbial load. This brief stationary phase was followed by a decrease in growth rate at 12.5g/l, as against 7.0g/l –11g/l.
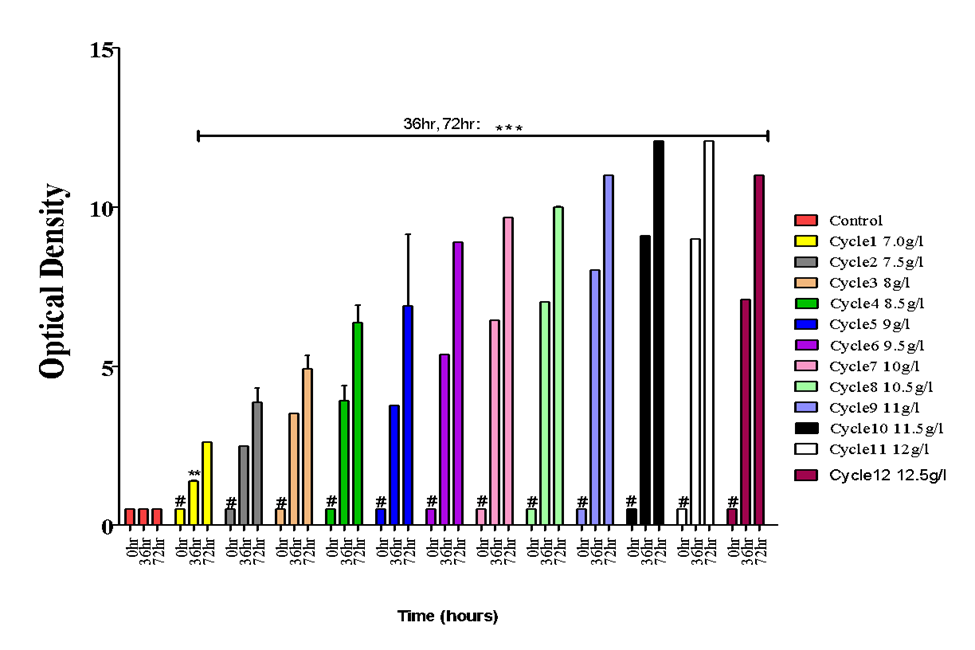
Figure 2: Adaptive Laboratory Evolution of Saccharomyces cerevisiae
Adaptive Laboratory Evolution. Results are given as mean value ± SD (n=5 Flasks). Vertical bars showing the hashtag symbols (#) are not statistically different from the control group, while bars with asterisk symbols (*) are statistically different from those in the control group. *p<0.05, ** p<0.01, ***p<0.001 vs control.
3.3 Quantitative PCR
Figure 3 shows the expression levels of targeted genes (PCK1, LSC1, ADH2 and ACS1) in wild and adapted isolates from cycles 7a (36 hours), 7b (72 hours), 10a (36 hours) and 10b (72 hours). An internal control gene (ACT1) was also adopted. The expression levels of ADH2 and LSC1 in 7b and 10b increased in comparison to the wild strain, and expression in 7a only slightly increased. 10a, on the other hand, had a reduced expression. PCK1 levels in 7a and 7b also increased compared to the wild strain. However, its expression was downregulated in 10a and 10b. ACS1 shows no visible increase in expression as against the wild strain, except in 7b.
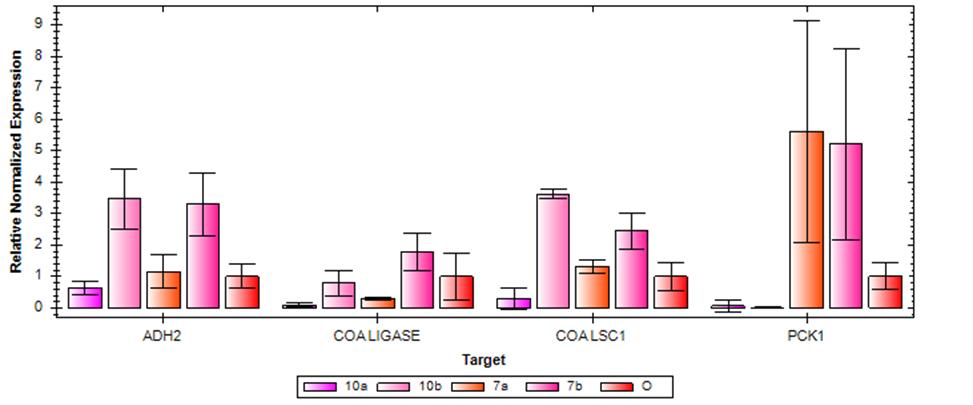
Figure 3: Relative Expression of Target Genes in Wild and Adapted Strains
4. Discussion
In this study, we adapted a wild yeast strain for increased ethanol tolerance. The wild strain had been isolated from spontaneous fermentation of banana wine and identified to be S. cerevisiae in a previous study. Upon treatment with different ethanol concentrations, the isolate was found to be least tolerant at 7% ethanol. Although, contrary to this, some studies have shown that an average S. cerevisiae strain is tolerant to ethanol at higher concentrations, other studies have revealed the existence of S. cerevisiae strains with tolerance levels as low as 6% and attributed the variations of ethanol tolerance level in different strains of S. cerevisiae to their membrane structure. (De Castro et al., 2011; Lairón-Peris et al., 2021)
Via ALE, across 12 generations, the tolerance level of the strain was successfully increased from 7% to 11.5%. The adapted strain was isolated at different generations, resulting in variants with different tolerance levels. The expression studies of selected target genes (identified in literature to be associated with ethanol tolerance) in the variants and wild strain shows that in comparison to the wild strain, ADH2 and LCS1 were upregulated in 7b and 10b. This forms a pattern, seen as the upregulation majorly occurred in cycle b of the 7th and 10th generation. This pattern could be attributed to better adaptation due to repeated cycles. The upregulation suggests that as a survival mechanism, the isolates utilised ethanol as a carbon source. This throws more light on their mode of adaptation and agrees with the work of De Smith et al. (2012) and Gasmi et al. (2014), which supports that a shift can occur from fermentation to respiration when glucose is scarce, and this shift results in reprogramming of gene expression. However, some other studies have shown that ethanol tolerance is a complex phenotype, and adaptation can also occur via other mechanisms, such as an increase in the biosynthesis of some amino acids, such as tryptophan (Yoshikawa et al., 2009) and trehalose accumulation (Bandara et al., 2009).
5. Conclusion
Using Adaptive Laboratory Evolution, in this research, the wild S. cerevisiae strain has been biotechnologically improved successfully, increasing its functionality in ethanol production. The study of the genetic expression of the adapted strains has shed light on the genetic modifications that occurred due to the ethanol stress conditions during adaptive laboratory evolution. Altogether, the findings of this study, if strategically channelled, could be highly instrumental in soothing the tension of alcohol production in the food industry.
This research has contributed to the knowledge of enhanced ethanol production via adaptation of the wild strain of S. cerevisiae.
Conflict of Interest
The authors declare that there were no conflicts of interest throughout the conduct of this study.
References
Araya, C. L., Payen, C., Dunham, M. J., & Fields, S. (2010). Whole-genome sequencing of a laboratory-evolved yeast strain. BMC genomics, 11, 1–10.
Avrahami-Moyal, L., Engelberg, D., Wenger, J. W., Sherlock, G., & Braun, S. (2012). Turbidostat culture of Saccharomyces cerevisiae W303-1A under selective pressure elicited by ethanol selects for mutations in SSD1 and UTH1. FEMS yeast research, 12(5), 521-533.
Bandara, A., Fraser, S., Chambers, P. J., & Stanley, G. A. (2009). Trehalose promotes the survival of Saccharomyces cerevisiae during lethal ethanol stress, but does not influence growth under sublethal ethanol stress. FEMS yeast research, 9(8), 1208–1216.
Chang, S. L., Lai, H. Y., Tung, S. Y., & Leu, J. Y. (2012). Dynamic large-scale chromosomal rearrangements fuel rapid evolution in yeast populations. PLoS Genet. 9 (1), e1003232.
Chiranjeevi T., Osuru H.P., Atluri N., Veeraraghavulu P., Raghavulu V., Yellapu N., Shaik M., Prasad U., Aluru S., Varma N., & Bhaskar, M. (2013). Hypothesis Isolation and characterisation of ethanol tolerant yeast strains.
Choe, D., Lee, J. H., Yoo, M., Hwang, S., Sung, B. H., Cho, S., … & Cho, B. K. (2019). Adaptive laboratory evolution of a genome-reduced Escherichia coli. Nature communications, 10(1), 935.
De Castro, P. A., Savoldi, M., Bonatto, D., Barros, M. H., Goldman, M. H. S., Berretta, A. A., and Goldman, G. H. (2011). Molecular characterisation of propolis-induced cell death in Saccharomyces cerevisiae. Eukaryotic Cell, 10(3), 398-411.
Dettman, J. R., Rodrigue, N., Melnyk, A. H., Wong, A., Bailey, S. F., & Kassen, R. (2012). Evolutionary insight from whole‐genome sequencing of experimentally evolved microbes. Molecular ecology, 21(9), 2058-2077.
Djegui, K. Y., Gachomo, E. W., Hounhouigan, D. J., Kayodé, A. P., & Kotchoni, S. O. (2014). Biochemical characterisation and growth patterns of new yeast isolates. Molecular biology reports, 41, 5199-5206.
Gasmi, N., Jacques, P. E., Klimova, N., Guo, X., Ricciardi, A., Robert, F., & Turcotte, B. (2014). The switch from fermentation to respiration in Saccharomyces cerevisiae is regulated by the Ert1 transcriptional activator/repressor. Genetics, 198(2), 547-560.
Good, B. H., McDonald, M. J., Barrick, J. E., Lenski, R. E., & Desai, M. M. (2017). The dynamics of molecular evolution over 60,000 generations. Nature, 551(7678), 45-50.
Gresham, D., & Dunham, M. J. (2014). The enduring utility of continuous culturing in experimental evolution. Genomics, 104(6), 399–405.
LaCroix, R. A., Palsson, B. O., & Feist, A. M. (2017). A model for designing adaptive laboratory evolution experiments. Applied and Environmental Microbiology, 83(8), e03115-16.
Lairón-Peris, M., Routledge, S. J., Linney, J. A., Alonso-del-Real, J., Spickett, C. M., Pitt, A. R., … & Querol, A. (2021). Lipid composition analysis reveals mechanisms of ethanol tolerance in the model yeast Saccharomyces cerevisiae. Applied and environmental microbiology, 87(12), e00440-21.
Liu, R., Bassalo, M. C., Zeitoun, R. I., & Gill, R. T. (2015). Genome-scale engineering techniques for metabolic engineering. Metabolic engineering, 32, 143–154.
Mattenberger, F., Fares, M. A., Toft, C., & Sabater-Muñoz, B. (2021). The role of ancestral duplicated genes in adaptation to growth on lactate, a non-fermentable carbon source for the yeast Saccharomyces cerevisiae. International Journal of Molecular Sciences, 22(22), 12293.
Ottilie, S., Luth, M. R., Hellemann, E., Goldgof, G. M., Vigil, E., Kumar, P., … & Winzeler, E. A. (2022). Adaptive laboratory evolution in S. cerevisiae highlights role of transcription factors in fungal xenobiotic resistance. Communications Biology, 5(1), 128.
Packer, M. S., & Liu, D. R. (2015). Methods for the directed evolution of proteins. Nature Reviews Genetics, 16(7), 379–394.
Shendure, J., Balasubramanian, S., Church, G. M., Gilbert, W., Rogers, J., Schloss, J. A., & Waterston, R. H. (2017). DNA sequencing at 40: past, present and future. Nature, 550(7676), 345-353.
Smets, B., Ghillebert, R., De Snijder, P., Binda, M., Swinnen, E., De Virgilio, C., & Winderickx, J. (2010). Life in the midst of scarcity: adaptations to nutrient availability in Saccharomyces cerevisiae. Current genetics, 56, 1-32.
Stanley, D., Fraser, S., Chambers, P. J., Rogers, P., & Stanley, G. A. (2010). Generation and characterisation of stable ethanol-tolerant mutants of Saccharomyces cerevisiae. Journal of Industrial Microbiology and Biotechnology, 37(2), 139–149.
Thornton, B., & Basu, C. (2011). Real‐time PCR (qPCR) primer design using free online software. Biochemistry and molecular biology education, 39(2), 145–154.
Xu, C., Sun, T., Li, S., Chen, L., & Zhang, W. (2018). Adaptive laboratory evolution of cadmium tolerance in Synechocystis sp. PCC 6803. Biotechnology for biofuels, 11, 1-15.
Yoshikawa, K., Tanaka, T., Furusawa, C., Nagahisa, K., Hirasawa, T., & Shimizu, H. (2009). Comprehensive phenotypic analysis for identification of genes affecting growth under ethanol stress in Saccharomyces cerevisiae. FEMS yeast research, 9(1), 32-44.
About this Article
Cite this Article
APA
Adeleke, D. F. & Gabriel-Ajobiewe, R. A. O. (2025). Optimization of Saccharomyces Cerevisiae for Increased Ethanol Tolerance via Adaptive Laboratory Evolution. In Akinyele B.J., Kayode R. & Akinsemolu A.A. (Eds.), Microbes, Mentorship, and Beyond: A Festschrift in Honour of Professor F.A. Akinyosoye. SustainE
Chicago
Adeleke, D. F. and Gabriel-Ajobiewe, R. A. O. 2025. “Optimization of Saccharomyces Cerevisiae for Increased Ethanol Tolerance via Adaptive Laboratory Evolution.” In Microbes, Mentorship, and Beyond: A Festschrift in Honour of Professor F.A. Akinyosoye, edited by Akinyele B.J., Kayode R. and Akinsemolu A.A., SustainE.
Received
15 November 2024
Accepted
10 January 2025
Published
4 February 2025
Corresponding Author Email: babatunde.kelly@aaua.edu.ng
Disclaimer: The opinions and statements expressed in this article are the authors’ sole responsibility and do not necessarily reflect the viewpoints of their affiliated organizations, the publisher, the hosted journal, the editors, or the reviewers. Furthermore, any product evaluated in this article or claims made by its manufacturer are not guaranteed or endorsed by the publisher.
Distributed under Creative Commons CC-BY 4.0
Share this article
Use the buttons below to share the article on desired platforms.